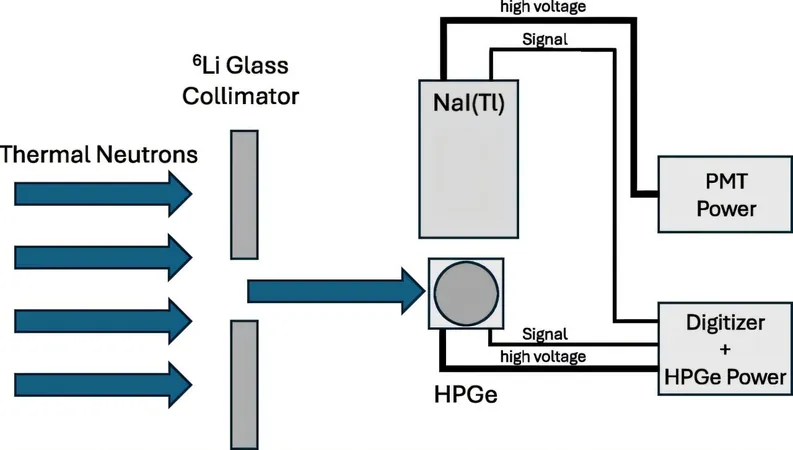
Groundbreaking Experiment Radically Boosts Detector Sensitivity to Neutrinos and Dark Matter by 50%!
2024-11-04
Author: Jacques
Introduction
In a remarkable advancement for the field of particle physics, researchers at the University of Michigan have fine-tuned an experimental setup that significantly enhances a detector's ability to sense neutrinos and potentially dark matter—two of the universe’s most elusive components. This pivotal study, published in *Physical Review D*, sheds light on the complex nature of these phenomena, which remain crucial for understanding the fabric of our universe.
Understanding Neutrinos and Dark Matter
Neutrinos are incredibly small, subatomic particles that are notoriously difficult to detect due to their weak interaction with other matter, a property that has earned them the label of 'ghost particles.' These minute particles are produced during nuclear reactions within stars and from radioactive decay. Remarkably, they barely interact with electromagnetic forces and have a mass about ten million times less than that of an electron, making their detection a true challenge for scientists.
Traditionally, neutrinos can only be detected via nuclear recoil—an event that occurs when a neutrino collides with an atomic nucleus. Such collisions are exceptionally rare; the event causes the nucleus to recoil, displacing nearby electrons. By applying a voltage across specialized detectors and measuring the resulting ionization energy, researchers can identify these rare neutrino-induced incidents.
Similarly, dark matter eludes direct detection, as it does not interact with light in any observable way. Scientists have theorized that dark matter, which is thought to account for approximately 27% of the universe’s mass, acts as a gravitational force holding galaxies together. Meanwhile, hypothesized particles known as Weakly Interacting Massive Particles (WIMPs) are believed to create effects similar to those of neutrinos and are a primary target in the search for dark matter.
Advancements in Detection Technology
Previously, detecting these phenomena required massive underground facilities, but advancements in technology now allow the use of compact, commercially available high-purity germanium (HPGe) detectors. These small detectors, measuring only a few inches long, exhibit enhanced sensitivity to weak nuclear recoils by utilizing the dense nucleus of germanium and operating at a frigid temperature of 77 Kelvin (around -196 °C). This extreme cooling mitigates atomic vibrations that could interfere with sensitive measurements.
To ensure accurate detection, researchers calibrated their techniques by assessing the response of germanium nuclei to low-energy nuclear recoils produced by neutron beams, a process that has been poorly understood in previous research. Igor Jovanovic, a U-M professor of nuclear engineering and the senior author of the study, emphasized the importance of understanding radiation interactions with matter, stating, 'Radiation is the means by which we observe the universe... Understanding how that radiation interacts with matter greatly impacts our ability to interpret measured results.'
Experimental Approach and Results
In the experimental setup, a HPGe detector measuring two cubic centimeters in size was paired with an external sodium iodide (NaI) scintillation detector to analyze the ionization effects from nuclear recoils. By saving raw data outputs from both detectors with advanced digital recording technology, the researchers could avoid biases from signal processing and apply multiple analysis algorithms for optimal results.
The findings from these experiments yielded a striking 50% increase in ionization yield compared to previous theoretical expectations. This leap forward enhances the sensitivity of HPGe detectors, presenting an unparalleled opportunity for more accurate detection of neutrinos and dark matter in ongoing experiments.
Implications and Future Directions
As lead author Alexander Kavner noted, 'Our results could greatly improve the sensitivity of commercially available detector technologies for neutrino detection and impact the results of several ongoing neutrino experiments.' This breakthrough paves the way for deeper investigations into the universe's structural mysteries and could redefine our understanding of fundamental physics.
The experimentation took place at The Ohio State University Nuclear Reactor Laboratory, emphasizing the collaborative efforts in this groundbreaking research initiative that promises to unravel the complexities of matter and the universe itself.